
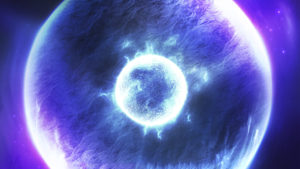
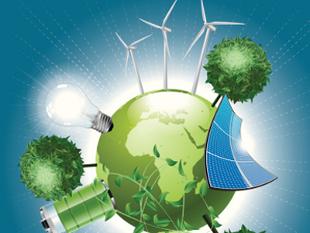
The Earth's Electrical Environment (Free Executive Summary) http://www.nap.edu/catalog/898.html
Free Executive Summary
The Earth's Electrical Environment
Geophysics Study Committee, Geophysics Research Forum, Commission on Physical Sciences, Mathematics and Resources, National Research Council
ISBN: , 263 pages, 8.5 x 11, hardback (1986)
This free executive summary is provided by the National Academies as part of our mission to educate the world on issues of science, engineering, and health. If you are interested in reading the full book, please visit us online at http://www.nap.edu/catalog/898.html . You may browse and search the full, authoritative version for free; you may also purchase a print or electronic version of the book. If you have questions or just want more information about the books published by the National Academies Press, please contact our customer service department toll-free at 888-624-8373.
This latest addition to the Studies in Geophysics series explores in scientific detail the phenomenon of lightning, cloud, and thunderstorm electricity, and global and regional electrical processes. Consisting of 16 papers by outstanding experts in a number of fields, this volume compiles and reviews many recent advances in such research areas as meteorology, chemistry, electrical engineering, and physics and projects how new knowledge could be applied to benefit mankind.
This executive summary plus thousands more available at www.nap.edu.
Copyright © National Academy of Sciences. All rights reserved. Unless otherwise indicated, all materials in this PDF file are copyrighted by the National Academy of Sciences. Distribution or copying is strictly prohibited without permission of the National Academies Press http://www.nap.edu/permissions/ Permission is granted for this material to be posted on a secure password-protected Web site. The content may not be posted on a public Web site.
OVERVIEW AND RECOMMENDATIONS 1
OVERVIEW AND RECOMMENDATIONS
INTRODUCTION
How does atmospheric electricity affect man and his technological systems? Is our electrical environment changing as a result of air pollution, the release of radioactive materials, the construction of high-voltage power lines, and other activities? It is clear that modern technological advances can be seriously affected by various atmospheric electrical processes and that man is also beginning to affect the electrical environment in which he resides. Our need to assess these technological and environmental impacts requires a better understanding of electrical processes in the Earth's atmosphere than we now possess. Further research is needed to understand better the natural electrical environment and its variability and to predict its future evolution.
We live in an environment that is permanently electrified. Certainly, the most spectacular display of this state occurs during intense electrical storms. Lightning strikes the Earth 50 to 100 times each second and causes the death of hundreds of people each year. Lightning is also a major cause of electric power outages, forest fires, and damage to communications and computer equipment; and new sophisticated aircraft are becoming increasingly vulnerable to possible lightning damage. Lightning contributes to the production of fixed nitrogen in the atmosphere, a gas that is essential for the growth of plants, and other trace gases. It is well known that the intense electric fields that are produced by thunderstorms can cause a person's hair to stand on end and produce corona discharges from antennas, trees, bushes, grasses, and sharp objects; these fields may also affect the development of precipitation in thunderstorms. Even in fair weather, there is an electric field of several hundred volts per meter near the ground that is maintained by worldwide thunderstorm activity.
In the Earth's upper atmosphere near 100-km altitude, a current of a million amperes flows in the high-latitude auroral zones; changes in the upper atmosphere currents, through electromagnetic induction, cause telluric currents to flow within power
OVERVIEW AND RECOMMENDATIONS 2
and communication lines as well as within the Earth and oceans. The upper atmospheric current systems are highly variable and are strongly related to solar-terrestrial disturbances. Power failures and communication disruptions have occurred during intense geomagnetic storms. It also appears that the electromagnetic transients that are produced by lightning and man-made power systems can affect trapped particle populations in the magnetosphere and cause particle precipitation into the upper atmosphere at low geomagnetic latitudes.
The practical needs for understanding many of the basic questions about atmospheric electricity were brought into clear focus on November 14, 1969. Thirty-six seconds after lift-off from the NASA Kennedy Space Center, Apollo 12 was struck by lightning, and 16 seconds later it was struck again. The first discharge disconnected all the fuel cells from the spacecraft power busses, and the second caused the inertial platform in the spacecraft guidance system to tumble. Fortunately, the rocket was still under control of the Saturn V guidance system at the time of the strikes; and, as a result, the astronauts, who had never practiced for such a massive electrical disturbance, were able to reset their circuit breakers, reach Earth orbit, realign their inertial platform, and ultimately land on target on the Moon. Although permanent damage to Apollo 12 was minimal, the potential for disaster of this lightning incident called attention to the important unanswered questions regarding lightning and atmospheric electricity.
Research in atmospheric electricity traditionally has been divided into several broad areas: (1) ion physics and chemistry, (2) cloud electrification, (3) lightning, (4) fairweather electrical processes, (5) ionospheric and magnetospheric current systems, and (6) telluric current systems. Most of this research has been pursued independently by scientists and engineers in different disciplines such as meteorology, physics, chemistry, and electrical engineering.
This study reviews the recent advances that have been made in these independent research areas, examines the interrelations between them, and projects how new knowledge could be applied for benefits to mankind. The study also indicates needs for new research and for the types of coordinated efforts that will provide significant new advances in basic understanding and in applications over the next few decades. It emphasizes a need to consider the interactions between various atmospheric, ionospheric, and telluric current systems that will be necessary to achieve an overall understanding of global electrical phenomena.
Lightning
Lightning is a large electric discharge that occurs in the atmosphere of the Earth and other planets and can have a total length of tens of kilometers or more. The continental United States receives about 40 million cloud-to-ground (CG) lightning strikes each year; on average, there are probably 50 to 100 discharges each second throughout the world (Chapter 1). Most lightning is produced by thunderclouds, and well over half of all discharges remain within the clouds. Most of our knowledge about the physics of lightning has come from the study of CG discharges. Most CG flashes effectively lower negative charge to ground, however recent evidence shows that positive charge can also be lowered (see Chapter 3 on positive lightning). Cloud-to-ground lightning kills about a hundred people and causes hundreds of millions of dollars in property damage each year in the United States; it is clearly among the nation's most severe weather hazards.
Most CG discharges begin within the cloud where there are large concentrations of positive and negative space charge (see Chapter 8). After several tens of milliseconds, the preliminary cloud breakdown initiates an intermittent, highly branched discharge that propagates horizontally and downward and that is called the stepped-leader (Figure 1). When the tip of any branch of the stepped-leader gets close to the ground, the large electric field that is produced near the surface causes one or more upward propa
OVERVIEW AND RECOMMENDATIONS 3
gating discharges to form. When an upward discharge makes contact with the steppedleader, the first return stroke begins. The return stroke is an intense wave of ionization that starts at or just above the ground and that propagates up the leader channel at about one third the speed of light. The return stroke is typically the brightest phase of lightning. The peak currents in these return strokes can reach several hundred thousand amperes; a typical value is about 40,000
- The peak electric power that is dissipated by a return stroke is on the order of 100 million watts per meter of channel; and the peak channel temperatures approach 30,000 K. A shock wave is produced by the rapid expansion of the hot, high-pressure channel, and this eventually becomes thunder with its own characteristics that depend on the nature of the discharge and the atmospheric environment (see Chapter 4).
Figure 1 The top figure shows the stepped-leader channel just before attachment; the bottom figure shows attachment and the development of the return stroke. The estimated time between the two figures is on the order of
0.001 sec.
The currents in the return stroke carry the ground potential upward and effectively neutralize most of the leader channel. After a pause of 40 to 80 milliseconds, most CG flashes produce a new leader, the dart leader, that propagates down the previous return-stroke channel and initiates a subsequent return stroke. Most flashes contain two to four return strokes, with each affecting a different volume of cloud charge (see Chapter 8
OVERVIEW AND RECOMMENDATIONS 4
). If a dart leader forges a different path to ground than the previous stroke, then the lightning will actually strike the ground in more than one place and will have a forked appearance. Chapters 2 and 3 discuss the physics of lightning in greater detail.
Among the more important advances that have been made in recent years has been the discovery that both in- cloud and CG discharges produce very fast-rising currents, i.e., rise times of tens to hundreds of nanoseconds and rates of change of current (dI/dt) on the order of 1011 A/sec. Most of the standard waveforms that are used to test the performance of lightning protectors and the integrity of lightning protection systems currently have current rise times and dI/dts that are substantially slower than the above values; therefore, these standards may not be an adequate simulation of the true lightning threat to aircraft and other structures (see Chapter 5).
A variety of nonequilibrium trace gases are produced within high-temperature lightning channels and by the shock wave that can affect tropospheric and stratospheric chemistry (see Chapter 6).
Recent spacecraft observations have shown that lightning may be present in the atmospheres of Jupiter, Venus, and Saturn; the upcoming Galileo probe will carry a lightning detector to Jupiter. In the future a study of lightning in atmospheres that are radically different from the Earth's may lead to a better understanding of the formation and characteristics of lightning on Earth.
Cloud Electrification
Although the vast majority of terrestrial clouds form and dissipate without ever producing precipitation or lightning, they can be weakly electrified. In some clouds, the electrification intensifies as convective activity increases, and strong electrification usually begins when there is rapid vertical and horizontal growth of the cloud and the development of precipitation. Most lightning on Earth is produced by cumulonimbus clouds that are strongly convective (i.e., they contain a vigorous system of updrafts and downdrafts) and that contain both supercooled water and ice. A small fraction of warm clouds are also reported to produce lightning.
The updrafts and downdrafts and the interactions between cloud and precipitation particles act in some still undetermined manner to separate positive and negative charges within the cloud. These processes usually transfer an excess of positive charge to the upper portion of the cloud and leave the lower portion with a net negative charge. Recent research has shown that the negative charge is usually concentrated at altitudes where the atmospheric temperature is between – 10°C and – 20°C (i.e., 6 to 8 km above sea level in summer thunderstorms and 1 to 3 km in winter storms) and that this altitude remains constant as the storm develops. This finding sets important criteria that must be met by any proposed thunderstorm charging mechanisms. The positive charge that is above the negative may be spread through deeper layers and does not exhibit as clear a relationship with temperature as does the negative charge. Positive charges are found at levels between – 25°C and – 60°C depending on the size of the storm, and this temperature range usually lies between 8 and 16 km above sea level.
Cloud electrification processes can be viewed as acting over two spatial scales: a microscale separation that ultimately leads to charged ice and water particles and then a larger-scale separation that produces large volumes of net positive and negative charge and eventually lightning. The microscale separation includes the creation of ion pairs, ion attachment, and charge that may be separated by collisions between individual cloud and precipitation particles. The larger cloud-scale separation may be due to precipitation or large-scale convection or some combination of the two.
Numerous mechanisms have been proposed for the electrification of clouds and thunderstorms, and several of these might be acting simultaneously. Feedback can occur through changes in the ion concentrations and electric field, and thus it is diffi
OVERVIEW AND RECOMMENDATIONS 5
cult to identify or evaluate the primary causes of electrification in a cloudy environment. Currently, there is a great need for more measurements to determine the locations, magnitudes, and movements of space charges within and near the cloud boundary. There is also a need to determine the charge-size relationship that is present on both cloud and precipitation particles, how these charges evolve as a function of time, and how these distributions are affected by lightning. Laboratory experiments have provided valuable information about the physics of selected microscale processes and are expected to continue to provide important data on the relative magnitude of various processes. Theory and numerical models also have played an important role in simulating and evaluating possible charging mechanisms on both the microscale and the cloud scale.
During the early nonprecipitating cloud stage, charging can occur by diffusion, drift, and selective capture of ions. Later, during the rain stage, there can be additional electrification due to drop breakup and other mechanisms based on electrostatic induction. Drift, selective ion capture, breakup, and induction are probably responsible for the charges and fields that are found in stratiform clouds; however, it is difficult to explain with just these mechanisms the stronger electrification that is found in convective clouds more than a few kilometers deep. For clouds in the hail stage, thermoelectric and interface charging mechanisms can provide strong electrification on the microscale.
In thunderclouds, the charges that are generated on a microscale can be subsequently separated on a larger cloud scale by convection and/or gravitational settling. Particles near the boundary of the cloud will become electrified by ion attachment, and the convection of these charges may play an important role in the electrification. Convection also plays a role in the formation and growth of cloud particles by forcing the condensation of water vapor until the particles are large enough to coalesce. Interactions between cloud particles, particularly when there are rebounding collisions, may also produce charge separation. If the larger particles tend to carry charge of predominantly one sign, they will fall faster and farther with respect to the convected air and leave the oppositely charged, smaller particles at higher altitudes.
As the populations of charged particles increase, the mechanisms that discharge these particles become more effective. Two kinds of discharging are possible: (1) discharge by ionic conduction, point discharge, or lightning and
- discharge by collision and/or coalescence with cloud particles of opposite polarity. The attachment of ions to cloud particles will be a function of the particle charge and the electric field of the cloud, and strong fields may also produce corona discharges from large water drops and the corners of ice crystals. Corona ions and lightning will increase the local electrical conductivity, and this, in turn, may prevent or reduce any further buildup of space charge in this region of the
Collisional discharge will take place at all stages of cloud particle growth. These mechanisms are enhanced if the interacting particles are highly charged and of opposite polarity; therefore, if a charging mechanism is to be effective, it must separate charge at a rate that is sufficiently high to overcome the discharging processes. It is worth noting that the electric forces on charged elements of precipitation can be several times larger than gravity; therefore, the terminal velocities and frequency of collisions of these particles will be a function of the electric field. More detailed discussions of the various processes involved in cloud electrification are given in Chapters 8, 9, and 10.
Recently, there have been attempts to analyze the patterns of the Maxwell current density that thunderclouds produce at the ground in order to define better the characteristics of the cloud as an electrical generator. The Maxwell, or total current density, contains components due to ohmic and non-ohmic (corona) ion conduction, convection, precipitation, displacement, and the charge-separating currents within the cloud. Under some conditions, there is evidence that the total current density may be
OVERVIEW AND RECOMMENDATIONS 6
coupled directly to the meteorological structure of the storm and/or the storm dynamics, but the lack of simultaneous Maxwell current measurements both on the ground and aloft has not allowed the details of this relationship to be determined. Such measurements will also be complicated by the complexity of the meteorological and electrical environment outside the storm.
Electrical Structure of the Atmosphere
It has been known for over two centuries that the solid and liquid Earth and its atmosphere are almost permanently electrified. The surface has a net negative charge, and there is an equal and opposite positive charge distributed throughout the atmosphere above the surface. The fair-weather electric field is typically 100 to 300 V/m at the surface; there are diurnal, seasonal, and other time variations in this field that are caused by many factors. The atmosphere has a finite conductivity that increases with altitude; this conductivity is maintained primarily by galactic cosmic-ray ionization. Near the Earth's surface, the conductivity is large enough to dissipate any field in just 5 to 40 minutes (depending on the amount of pollution); therefore, the local electric field must be maintained by some almost continuous current source.
Ever since the 1920s, thunderstorms have been identified to be the dominant generator in the global circuit. Most cloud-to-ground lightning transfers negative charge to the ground, and the point discharge currents under a storm transfer positive space charge to the atmosphere. In addition, there are precipitation and other forms of convection currents and both linear and nonlinear conduction currents that must be considered when attempting to understand the charge transfer to the earth by a thunderstorm. The electrical structure of a thunderstorm is complex (see Chapter 8), but it is often approximated simply as a vertical electric dipole.
The conductivity of the fair-weather atmosphere near the surface is on the order of 10-14 mho/m, and it increases nearly exponentially with altitude to 60 km with a scale
Figure 2 Schematic of various electrical processes in the global electrical circuit.
OVERVIEW AND RECOMMENDATIONS 7
height of about 6 km. The main charge carriers below about 60 km are small positive and negative ions that are produced primarily by galactic cosmic rays. Above 60 km, free electrons become more important as charge carriers and their high mobility produce an abrupt increase in conductivity throughout the mesosphere. Above 80 km, the conductivity becomes anisotropic because of the influence of the geomagnetic field, and there are diurnal variations due to solar photoionization processes.
Figure 3 Nomenclature of atmospheric regions based on profiles of electrical conductivity (σ), neutral temperature, and electron number density.
The atmospheric region above about 60 km is known as the equalization layer and is usually assumed to be an isopotential surface and the upper conducting boundary of the global circuit. Currents flow upward from the tops of thunderstorms to this layer where they are rapidly distributed throughout the world. Worldwide thunderstorms maintain a potential difference of 200 to 600 k V between the equalization layer and the surface—the Earth-ionosphere potential. This potential difference, in turn, drives a downward conduction current that is on the order of 2 × 10-12 A/ m2 in fair-weather regions and constant with altitude.
Today, there are still many details that need to be clarified about the role of thunderstorms as the generators in the global circuit (Figure 2). Upward currents have been detected above thunderclouds, but how these currents depend on storm dynamics, stage of development, lightning frequency, precipitation intensity, and cloud height, for example, is still not known. There is a need for further measurements to quantify the relationships between diurnal variations of the ionospheric potential, the electric field or air-earth current, and worldwide estimates of thunderstorm frequency.
Many electrical processes interact within the global circuit, and the following subsections will describe selected processes that occur within certain atmospheric regions (Figure 3). It should be recognized that the global circuit includes mutual electrical interactions between all atmospheric regions.
OVERVIEW AND RECOMMENDATIONS 8
This report will not present an encyclopedic review of all the electrical phenomena that occur in the atmosphere but will simply give some examples that illustrate a few of the basic processes and some of the important interrelationships. Notably absent, but important to an overall understanding of atmospheric electricity, are discussions dealing with electromagnetic phenomena such as sferics, Schumann resonances, and whistlers. These and many other subjects, however, have been reviewed in the two-volume Handbook of Atmospherics, edited by Volland (1982), and recent proceedings of international conferences on atmospheric electricity (Dolezalek and Reiter, 1977; Orville, 1985). Human and biological effects of atmospheric electricity are also important research areas that are not considered in this study.
The Planetary Boundary Layer
The planetary boundary layer (PBL) is the lowest few kilometers of the atmosphere where interactions with the surface, man, and the biosphere are the most pronounced. Galactic cosmic rays are the main source of ionization in the PBL; however, near land surfaces, ionization is also produced by decays of natural radioactive gases emanating from the soil surface and by radiations emitted directly from the surface. Ionization from radioactive sources depends on soil type and surface structure and on the meteorological dispersal rate; this ionization normally decreases rapidly with altitude, and at about 1 km its contribution to the total ionization is less than that from cosmic rays. Other sources of ions in the PBL include lightning; electrification due to waterfalls, ocean surf, and man-made sprays; a variety of combustion processes; point discharge or corona currents that are produced whenever the ambient electric field exceeds breakdown; and frictional processes associated with blowing dust, snow, or volcanic ejecta.
In the troposphere, atmospheric trace gases are numerous and variable, and the ion chemistry is complicated by clustering processes and the relatively long lifetime of the terminal ions. As a further complication, clouds and other aerosols play an important role as sinks for small ions and thereby alter the ion distribution (see Chapter 11). Over continental areas, the loss of ions by attachment to aerosols can be larger than the loss by recombination. Some atmospheric aerosols are hygroscopic, and the particle size increases with relative humidity. At large humidities, fog and cloud droplets form and produce a large decrease in the electrical conductivity of the atmosphere. Since a decrease in conductivity can be a precursor of fog, it might be possible to improve forecasts of the onset of fog by electrical measurements.
Turbulent transport and convection within the PBL are important processes that govern the momentum, heat, and moisture exchanges between the atmosphere, geosphere, and biosphere. These processes influence the mean wind profile, the vertical distribution of temperature, water vapor, trace gases, aerosols, and the ion distribution throughout the troposphere. Turbulent mixing and convection can prevent the buildup of radioactive emanations near the ground and can also disperse aerosols to a greater altitude in the troposphere.
Electrical processes in the PBL are complex, highly variable, and span a tremendous range of space and time scales. The electrical variables respond to many of the lower atmospheric processes but usually have little influence on the phenomena to which they respond. Within the PBL, local turbulent fluctuations of space-charge density impose a time-varying electric field that is comparable in magnitude with or even greater than the electric field maintained by global thunderstorm activity. Since the PBL is the region of the atmosphere with the greatest resistance, it is this layer as well as the generators that control the currents in the global circuit. Electrical processes in the PBL are discussed in greater detail in Chapter 11.
OVERVIEW AND RECOMMENDATIONS 9
Mid-Troposphere-Stratosphere
The main source of ionization in the mid-troposphere-stratosphere (˜ 2-50-km) region is cosmic radiation; the ionization rate depends on magnetic latitude and on solar activity. At about 50° geomagnetic latitude and at 20-km altitude, the ion-production rate during sunspot maximum is about 30 percent smaller than during sunspot minimum; at 30-km altitude (same latitude) it is about 50 percent smaller. Following solar flares that produce energetic charged particles (solar proton events), the ion-production rate in the stratosphere may increase by orders of magnitude for periods of hours to days, but deeper in the atmosphere the effect is much smaller. Solar flares are usually followed by a reduction (Forbush decreases) in the ion-production rate for periods of hours to weeks that is caused by a temporary reduction of the incoming cosmic-ray flux.
The composition and chemistry of the ions that establish the bulk electrical properties in the mid-troposphere- stratosphere are relatively unknown. The ion concentrations are also affected by aerosols whose distributions are quite variable in both space and time. Aerosols tend to accumulate at temperature inversion boundaries and can cause a general loss of visibility that can be seen by airline passengers as they pass through such layers. Such a buildup of aerosols causes a general decrease in the smallion concentration, and, thus, the electrical conductivity is also reduced— resulting in an increase in the local electric field.
The concentration of particles with a radius greater than about 0.1 micrometer decreases with altitude above the PBL, and a relative minimum occurs in the upper troposphere. The particle concentrations increase within the lower stratosphere, peak near 20 km, and then decrease again with altitude. This persistent structure is frequently referred to as the 20-km sulfate layer; the character of this layer is controlled largely by gases emitted during volcanic eruptions, as discussed in Chapter 12.
Aerosol particles that have radii on the order of 0.01 micrometer are referred to as condensation nuclei (CN) and are uniformly mixed throughout the troposphere above the PBL. Near the surface, the CN concentration may be large owing to local sources; above the tropopause the concentration decreases with altitude. In recent years, a CN layer has frequently been observed near 30 km. As a result of the El Chichon volcanic eruption in 1982, the normal CN concentration at 30-km altitude increased by at least two orders of magnitude and measurably affected the ion concentration and electrical conductivity.
Under steady-state conditions, the air-earth current density is constant with altitude if there is large-scale horizontal homogeneity and if no thunderstorms or other localized electrical disturbances are in the vicinity. The air- earth current varies with magnetic latitude because of the magnetic variations in cosmic-ray fluxes. The current is generally enhanced over orographic features such as mountain ranges because of the decreased columnar resistance (mountains are closer to the ionosphere than the near-sea-level surface). Estimates have been made that indicate that as much as 20 to 30 percent of the total global current flows into the high mountain peaks.
Mesosphere
In the mesosphere (50-85 km altitude), the major daytime source of ionization is solar Lyman-alpha photoionization of nitric oxide (NO). The major source of NO for this region is the thermosphere, where NO is produced by extreme ultraviolet (EUV) radiation (wavelengths less than 100 nm) and auroral particle precipitation. Meteorological processes in the upper atmosphere transport NO from the thermosphere to the mesosphere, where its distribution is variable. Somewhat smaller sources of ionization in the upper mesosphere include solar x-ray ionization and the photoionization of oxygen in a metastable state. At high latitudes, energetic electrons, protons, and bremsstrahlung
OVERVIEW AND RECOMMENDATIONS 10
ultraviolet radiation associated with auroral particle precipitation are variable sources related to geomagnetic activity.
Solar protons are a sporadic and intense source of ionization at high latitude following intense solar flares. These solar proton events can increase the electrical conductivity of the magnetic polar-cap mesosphere by several orders of magnitude (at altitudes down to about 50 km) during such events. In addition, the current carried by the bombarding solar protons can often exceed the local air-earth conduction current flowing in the circuit.
The principal primary positive ions produced in the mesosphere are , , and NO+, but all participate in a wide range of reactions that lead to a rich spectrum of ambient positive ions. An equally rich range of negative ions is generated by reactions initiated by the attachment of electrons to form the main primary species, and O-. Rocketborne mass-spectrometer measurements have shown that below the mesopause the positive ions are proton hydrates with as many as 20 water molecules clustering to individual ions. The positive-ion chemistry of the mesosphere is better understood than is the negative-ion chemistry (see Chapter 13).
The interaction of the terminal ions with aerosol particles is probably a significant sink for ions in the polar aerosol layers near the summer mesopause where noctilucent clouds are commonly observed.
The electrical conductivity of the mesosphere is important because it governs the electrical properties of the equalization layer in the global circuit. Below about 60 km, the terminal small ions are the main charge carriers; but above 60 km, free electrons can exist and their high mobility is responsible for the abrupt increase in electrical conductivity observed in the mesosphere. Furthermore, above 70 km, collisions between electrons and air molecules become infrequent enough so that electrons are confined to spiral about a magnetic field line and the motion perpendicular to the field becomes more difficult than motion along the field. The electrical conductivity becomes anisotropic, and this anisotropy has a dominant influence on the electrical properties of the global circuit above 70 km.
Rocketborne measurements of the upper atmosphere conductivity and electric field indicate some puzzling features. There appear to be regions in the upper stratosphere and mesosphere that have abrupt increases and decreases in vertical conductivity profiles. The decreases are probably associated with aerosol layers, but the increases are difficult to interpret. On occasion, the electric field near 50to 70-km altitude has been observed to increase enormously from what is expected if the mesosphere is a passive element in the global circuit. The mesosphere may not be electrically passive but may, in fact, contain active electrical generators that are not currently known.
Ionosphere and Magnetosphere
The major sources of ionization above about 85 km are extreme-ultraviolet (EUV) radiation and auroral particle precipitation (see Chapter 14). The ionizing portion of the solar spectrum (i.e., wavelengths below 102.7 nm) is absorbed in the thermosphere and creates an ionosphere that consists of positive molecular and atomic ions (e.g., , NO+, , O+) and negative electrons. The solar EUV radiation and the electron and ion densities throughout the ionosphere are highly dependent on solar activity; there are known variations with the 11-yr sunspot cycle, the 27-day rotation of the Sun, and solar flares.
Auroral particle precipitation is responsible for large variations in ion and electron densities at high latitudes. The bulk of the precipitation occurs within the auroral oval that encircles the geomagnetic pole in magnetic conjugate polar caps. Observations over many years show that there is always auroral activity within the oval. The activity varies considerably over the day and even from hour to hour owing to interactions of
OVERVIEW AND RECOMMENDATIONS 11
the solar-wind plasma with the Earth's magnetic field. The total power dissipated by particles bombarding the upper atmosphere is typically 109 W, but during large geomagnetic storms it can approach 1012 W.
The sources and composition of the ions that maintain the bulk electrical properties of the upper atmosphere are generally known on the dayside of the Earth, but at night there are still uncertainties with regard to the ionization sources.
In the classical view of the global circuit (see Chapter 15), the ionosphere is assumed to be at a uniform potential with respect to the surface; however, the known upper-atmosphere generators are not included. The two major generators that operate in the ionosphere above about 100 km are the ionospheric wind dynamo and the solar-wind/ magnetosphere dynamo (see Chapter 14). Atmospheric winds have the effect of moving the weakly ionized ionospheric plasma through the geomagnetic field. This movement produces an electromotive force and generates electric currents and fields. This process is complicated by the variability of the ionospheric winds and the anisotropic electrical conductivity in the ionosphere.
The magnitude of the horizontal electric field associated with the wind-driven dynamo is on the order of 1 m V/m. A total current of about 100,000 A flows horizontally in the ionosphere because of the combined action of the wind and electric field, mainly on the sunlit side of the Earth. This current flows in two counterrotating vortices on opposite sides of the equator, and these patterns dominate at low latitudes and midlatitudes. Global-scale horizontal potential differences of about 5 to 10 kV are generated by the ionospheric wind dynamo.
The ionospheric winds that drive the dynamo are mainly caused by upward propagating tides from the lower atmosphere that have large day-to-day fluctuations. During geomagnetic storms, however, thermospheric winds increase in response to high-latitude auroral heating and cause disturbances at low latitudes to the fields and currents of the ionospheric wind dynamo.
The solar-wind/magnetosphere dynamo results from the flow of the solar wind around and perhaps partly into and within the Earth's magnetosphere. The motion of this plasma through the geomagnetic field produces an electromotive force and currents at high latitudes that result in an antisunward flow of plasma over the magnetic polar cap and a sunward flow of ions in the vicinity of the dawn and dusk auroral zones. This motion is described by a two-cell counterrotating ion circulation with one cell on the dawn side and the other on the dusk side of the magnetic polar caps. The polar-cap electric field is typically 20 m V/m, with an ionospheric convection velocity of 300 m/sec. Larger fields of about 50 to 100 m V/m occur in the vicinity of the auroral ovals. The large-scale potential difference that is associated with this horizontal ion flow over the polar caps has a total dawn-to-dusk drop of about 50 kV. This potential drop and the configuration of the two-cell pattern are highly variable. The potential drop has values of 20 to 30 kV during geomagnetic quiet conditions that increase to 100 to 200 kV during geomagnetic storms. These fields are mainly confined to the polar caps because of the shielding from currents within the magnetosphere. During geomagnetic storms, however, the shielding currents can be altered and electric fields have been observed to propagate all the way from the polar caps to the equator.
Currents are an integral part of the complex electrical circuit associated with the solar-wind/magnetosphere dynamo. Currents flowing along the direction of the magnetic field couple the auroral oval and high-latitude ionosphere with outer portions of the magnetosphere. Typically about a million amperes of current flow in the solar- wind/magnetosphere dynamo. The dynamo currents and fields with this high-latitude system are extremely complex and highly variable (see Chapter 14).
The large-scale horizontal fields (scale sizes 100 to 1000 km) within the ionosphere can propagate or map downward in the direction of decreasing electrical conductivity. Horizontal fields of a smaller scale (1 to 10 km) on the other hand are rapidly attenu
OVERVIEW AND RECOMMENDATIONS 12
ated. The larger-scale horizontal electric fields that do map to the surface become vertical at the surface because of the high surface conductivity. The solar-wind/magnetosphere field can alter the surface fields at high latitudes by 20 to 50 V/m depending on the level of geomagnetic activity and the magnitude of the dawn-to-dusk potential drop across the magnetic polar cap.
Telluric Currents
Telluric currents consist of both natural and man-induced electric currents flowing in the solid earth and oceans. The fundamental causes of the natural currents are electromagnetic induction resulting from a time-varying, external geomagnetic field or the motion of a conducting body (such as seawater) across the Earth's internal magnetic field. These telluric currents, in turn, produce magnetic fields of their own that add to the external geomagnetic field and that produce a feedback on the ionospheric current system. The complexities associated with telluric currents arise from the complexities in the external current sources and the conductivity structure of the Earth (see Chapter 16).
The external inducing field also has various scale sizes that contribute to the complexities in the telluric current systems. The ionospheric dynamo currents that are associated with the solar diurnal and lunar tides have a planetary- scale size. The ionospheric current variations, however, also have smaller-scale features that are associated with auroral and equatorial electrojets. At low frequencies, the external inducing sources can be approximated by a planetary-scale field that is occasionally altered by strong spatial gradients during geomagnetically disturbed conditions. At higher frequencies (magnetic storms, substorms, or geomagnetic pulsations), the source can often be quite localized and highly time dependent.
Electromagnetic induction caused by ionospheric and magnetospheric current variations has a pronounced effect on telluric currents and on man-made systems. These effects have been detected by a number of investigators, and it is now well recognized that there is a direct electromagnetic coupling from the ionosphere to the telluric currents. The large variation in conductivity of the solid earth can give rise to various channeling effects within the Earth, thereby considerably complicating the flow patterns of the telluric currents. The current patterns are different for different frequencies of external induction. The longer the period of the time-varying field, the deeper into the Earth the induced currents are expected to flow. For example, a signal with a period of about 24 hours is generally believed to have a skin depth of 600 to 800 km. The distribution of sediments, the degree of hydration, differences in porosity, and other properties of the Earth all have an influence on the signal response. Properly interpreted, telluric currents can be a tool to study both shallow and deep structures within the Earth.
Techniques for Evaluating the Electrical Processes and Structure
The cornerstone of our understanding of the Earth's electrical environment is an integration of measurements, theory, and modeling. The new instruments and techniques that have been developed in recent years are diverse, and various chapters in this volume contain details on techniques beyond those illustrated in this Overview.
Remote measurements of electric and magnetic fields can now be used to infer many properties of lightning and lightning currents. Also, the amplitude and time characteristics of thunder and various radio-frequency (rf) noise emissions can be used to trace the geometrical development of lightning channels within clouds as a function of both space and time. There are now large networks of ground-based lightning detectors that
OVERVIEW AND RECOMMENDATIONS 13
can discriminate between in-cloud and cloud-to-ground lightning and accurately determine the locations of ground- strike points. With such a detection capability, it should now be possible to determine whether and how the characteristics of individual cloud-to-ground discharges depend on their geographic location, the local terrain, and/or the meteorological structure of the storm.
The rf noise that is generated by lightning in the hf and vhf bands appears in the form of discrete bursts, and within a burst there are hundreds to thousands of separate pulses. If the difference in the time of arrival of each pulse is carefully measured at widely separated stations, the location of the source of each pulse can be computed, and the geometrical development of the rf bursts can be mapped as a function of time.
Satellite observations of lightning have provided rough estimates of the global flashing rates and the geographic distribution of lightning as a function of season. Optical detectors, such as those now in orbit on the Defense Meteorological Satellite Program (DMSP) satellites, are limited in their temporal and spatial coverage, but they have provided data that show a progression of lightning activity toward the summer hemisphere and notable absences of lightning over the ocean during the observing intervals (see Chapter 1). The data to date are only for local midnight, dawn, and dusk; there is a need to obtain data at other times. Measurements of hf radio noise by the Ionosphere Sounding Satellite-B have also been used to estimate a global lightning flash rate.
Global detection of lightning is necessary to determine the global flashing rate and how this rate relates to other parameters in the global circuit. In recent years, the National Aeronautics and Space Administration has developed new optical sensors that could be used to detect and locate lightning in the daytime or at night and with continuous coverage by using satellites in geosynchronous orbits. These sensors are capable of measuring the spatial and temporal distribution of lightning over extended periods with good spatial resolution and offer significant new opportunities for research—without the inherent sampling biases of low-altitude orbiting satellites—and for many applications.
Artificial triggering of lightning now provides the capability of studying both the physics of the discharge process and the interactions of lightning with structures and other objects in a partially controlled environment (see Chapter 2). When a thunderstorm is overhead and the surface electric field is large, a small rocket is launched to carry a grounded wire rapidly upward. When lightning is triggered by the wire, the first stroke is not like natural lightning, but subsequent return strokes appear to be almost identical to their natural counterparts.
Triggered lightning is now being used to investigate the luminous development of lightning channels, the characteristics of lightning currents, the velocities of return strokes, the relationships between currents and electromagnetic fields, the mechanisms of lightning damage, the performance of lightning protection systems, and many other problems. The main benefit of this triggering technique is that it can be used to cause lightning to strike a known place at a known time, thus enabling controlled experiments to be performed. Although lightning cannot be reproduced in full in the laboratory, several lightning simulators have been developed and have provided some quantitative information on the generation of thunder.
Cloud electrification and charge-separation processes are closely coupled to the cloud microphysics and the storm dynamics. The natural storm environment is extremely complicated, and its quantification involves a host of electrical and meteorological parameters. Many of these parameters and their measurements are treated in Chapters 7 and 8 and the three-volume publication, Thunderstorms: A Social, Scientific, and Technological Documentary, edited by Kessler (1982). One of the greatest needs is for an in-cloud instrument that can measure in a thunderstorm environment the charge on the smaller cloud particles as a function of particle size and type (see Chapter 8).
OVERVIEW AND RECOMMENDATIONS 14
In recent years, cooperative field programs, such as the Thunderstorm Research International Program (TRIP), have improved our knowledge about the overall electrical structure of thunderstorms. These programs have also provided a framework wherein a number of different investigators using different techniques can study the same thunderstorms at the same location at the same time. For instance, in situ and remote electrical measurements have been made in New Mexico in conjunction with Doppler radar studies of the cloud precipitation and dynamics and in- cloud sampling of the larger cloud particles. Laboratory experiments also continue to provide information on the effects of electricity on cloud microphysics and charge separation mechanisms that are critical to the interpretation of data collected by such field programs. Finally, numerical models of the electrical development and structure of thunderstorms have provided an important framework in which to interpret the cloud measurements and laboratory experiments.
The techniques that are used to determine the electrical structure of the fair-weather atmosphere are diverse. Usually, vertical profiles of one or more atmospheric-electrical variables—typically electric field, conductivity, and current density—are measured over a relatively short time span. Sensors are carried aloft on aircraft, balloons, or rockets, and the data are presented both as profiles and as numerically integrated results. The vertical profiles represent almost instantaneously measured parameters rather than time averages. Aircraft or constant-pressure balloons, however, do have the capability of measuring temporal variations at a given level. Profiles have been measured over land because of the convenience and to study specific terrain effects and over water in attempts to eliminate distortions caused by land. Profiles have led to the detection of convection currents in the planetary boundary layer that are comparable in magnitude with the total current, the electrode effect over water under stable conditions, the response of columnar resistance to pollution, and the diurnal variation in ionospheric potential.
In addition to the standard electrical parameters, determinations of ion and aerosol contents and compositions, mobility, and chemistry are all critical to an understanding of conductivity. Again, these quantities are usually displayed as vertical profiles and, to a certain extent, are characteristic of the type of platform on which the instrumentation is carried aloft (e.g., some airplane measurements provide horizontal profiles but are limited in their vertical extent). Vertical measurements are probably a good first approximation to the global electrical structure; however, horizontal variations should also be measured as a function of time to complement the profile data. Tethered balloons can provide the time variations of selected electrical properties at a few locations, and this has been attempted on an experimental basis. For understanding the global circuit, it would be valuable to have a number of vertical profiles taken at the same time and, in addition, to repeat certain profiles to obtain the time variations.
Knowledge of the upper-atmosphere current systems is important for understanding the interactions among the ionosphere, magnetosphere, and the solar wind. Some of these current systems were studied during the International Magnetospheric Study, 1976-1980, and during the NASA Dynamics Explorer satellite program. The goals of these programs have been to investigate the coupling of the solar-wind energy through the magnetosphere and into the ionosphere; but little effort was made to couple these current systems into the global electrical circuit. Additional measurements of magnetosphere-ionosphere currents are planned for the International Solar-Terrestrial Physics program.
Societal Impact
Severe weather phenomena that disrupt our lives include tornadoes, hail, high winds, hurricanes, floods, snowstorms, and lightning. Among them, lightning ranks as
OVERVIEW AND RECOMMENDATIONS 15
the number one killer, followed closely by tornadoes. Lightning is much less dramatic than a tornado passing through an area or a severe snowstorm that paralyzes a city, but lightning can strike quickly and kill with little or no warning.
Lightning is a leading cause of outages in electrical power systems and was the initial cause of the massive power blackout in New York City on July 13, 1977. The possible effects of lightning on advanced aircraft, nuclear power stations, and sophisticated military systems are problems of increasing concern.
The detailed physics of how lightning strikes a structure, a power line, or an aircraft and its effects are still not known. The approaching leader is not influenced by the object that is about to be struck until it is perhaps a few tens of meters away. At that time, an upward-moving streamer leaves the object and similar discharges may also leave other objects nearby. When the upward-moving streamer attaches to the downward-moving leader, the return stroke begins. When the details of this attachment are better understood we should be able to predict with higher probability what will and what will not be struck under various conditions and thereby provide better lightning protection. For example, the positioning of overhead ground wires above power transmission lines and the protection of complex structures could be optimized (see Chapter 5).
The current rise time is an important parameter for lightning protection because if the current interacts with an inductive load, the voltage on that load is proportional to the rate of increase of the current. Most of the standard surge waveforms that are used to verify the performance of protectors on power and telecommunications circuits specify that open-circuit voltage should have a rise time of 0.5, 1.2, or 10 microseconds and that the short-circuit current should have a rise time of 8 or 10 microseconds. These values are substantially slower than recently measured lightning current rise times, which are in the range of tens to hundreds of nanoseconds; therefore, it is probable that the degree of protection that is provided by devices tested to present-day standards will not be adequate for protection against direct lightning surges.
The unusually destructive nature of lightning that lowers positive charge to ground is only partially documented and is poorly understood (see Chapter 3). Because of the large and long continuing currents, positive lightning may ignite a disproportionately large number of fires, especially in grasslands and forests. The apparent pattern is for positive lightning to strike preferentially outside areas of rainfall, and this further enhances the likelihood of its starting a fire. Positive lightning may be correlated with storm severity and tornado occurrence, and its detection could enhance our present severe-storm detection and warning systems.
Newly developed lightning-detection equipment now makes it possible to make real-time decisions on the preparations for repairs of utility systems, early warning and detection of lightning-caused forest fires, and a variety of other warning functions in situations that allow protective action to be taken, such as launches at the NASA Kenedy Space Center and outdoor recreational activities. Among the main users of lightning location data at present are the Bureau of Land Management (BLM) in the western United States and Alaska and the Electric Power Research Institute in the eastern United States. The BLM and the Forest Services of most Canadian provinces utilize the time and location of lightning storms to determine when and where to look for forest fires. Early detection of these fires provides considerable savings both in the natural resources and in the cost of fighting the fires. In the eastern United States the lightning data are being used to accumulate statistics on lightning occurrence and for real-time applications by electric power utilities. For warnings of lightning-intensive storms, these data are also disseminated in real time to many National Weather Service offices and to a growing number of television stations.
Although cloud electrification processes are ultimately responsible for producing lightning, these processes can also electrify an aircraft flying in a cloud. It is quite
OVERVIEW AND RECOMMENDATIONS 16
common for the potential of an aircraft to be raised by several million volts, and most planes have discharger wicks to control the interference in radio communications when the aircraft goes into point discharge. Lightning will not usually present a hazard to commercial aircraft as long as the present design practices are continued and the standard practice of avoiding large thunderclouds is maintained. However, since many new aircraft are being developed with composite materials instead of aluminum and with the increased use of computers and microcircuit technology, the decreased electrical shielding on the outside and increased sensitivity inside means that there will be an increasing vulnerability to lightning disturbances (see Chapter 5). Thus, cloud electricity and lightning will have to be considered carefully in the design and operation of future aircraft systems.
Natural telluric currents can significantly disturb man-made systems such as communication cables, power lines, pipelines, railways, and buried metal structures. The largest natural disturbances are associated with the intense auroral current systems that flow at high latitudes during geomagnetic storms. There have been frequent reports of these disturbances, inducing currents on long telephone and telegraph wires that are large enough to generate sparks and even permanent arcs. When this occurs, there can be outages and shutdowns in both land and sea cables and fires can be started by overheating the electrical systems. Currents of up to about 100 A are sometimes induced in power transformers at northern latitudes and cause power blackouts and system failures. During the large geomagnetic storm of February 11, 1958, the Toronto area suffered from an induced power blackout.
Long pipelines are also affected by telluric current disturbances. The Alaskan pipeline has been the subject of careful investigation, principally because of its location across the auroral zone. One of the concerns has been the rate of corrosion of the pipeline, which is enhanced by telluric currents. However, telluric currents appear to affect electronic equipment related to operational monitoring and corrosion control rather than to produce specific serious corrosion problems. A relationship between the expected current flow and geomagnetic activity has been derived and suggests that the pipeline is a large man-made conductor that is capable of significantly affecting the local natural regime of telluric currents.
There is also a concern that the long, power-transmission lines planned for future arctic development will be subject to larger induced currents by auroral activity than was previously considered. This would require new protection equipment development for high-latitude applications.
Telluric currents have also been used in the search for natural resources with two different approaches— magnetotellurics and geomagnetic depth sounding. Telluric currents can also be used to study long-period tidal phenomena and water flows and the Earth's astronomical motion and as possible precursors for earthquakes and volcanic eruptions. It has also been suggested that a natural waveguide for telluric currents in the Earth's crust, consisting of an insulating layer of dry rocks sandwiched between an upper hydrated conducting layer and an underlying conducting hot layer, could be used for communications. There are also investigations to determine the feasibility of using the natural resonances in the earth-ionosphere waveguide, Schumann resonances, as a means for long-distance communications.
Recommendations
An increased interest in understanding the Earth's electrical environment has resulted from recent advances in different disciplines, along with the recognition that many of man's modern technological systems can be adversely affected by this environment. This understanding appears to be on the threshold of rapid progress.
OVERVIEW AND RECOMMENDATIONS 17
There should be a concerted effort of coordinated measurement campaigns, supported by critical laboratory experiments, theory, and numerical modeling of processes, to improve our understanding of the Earth's electrical environment.
Because the study of the electrical environment is commonly divided into three major components—lightning, cloud electricity, and the global circuit (including ion chemistry and physics and ionospheric, magnetospheric, and telluric currents)—the specific needs in these areas are detailed below. However, it should not be forgotten that there are interactions among these components and that the understanding of these interactions may be fundamental to an understanding of an individual component.
- More needs to be known about the basic physics of the lightning discharge and its effects on structures in order to design proper protection systems. Most lightning begins within a thundercloud, but the initiation and subsequent development of a flash within the cloud are poorly understood. The physics of electrical breakdown over distances of 10-10,000 m is not understood, nor is the relationship between the channel geometry and the fields and charges that existed before the discharge. The fields and currents that are produced by most of the important lightning processes have large submicrosecond variations, but how the discharge currents develop as a function of space and time and what the ranges of variability of the maximum I and dI/dt parameters are need to be determined. The power and energy balances within the lightning channel and many other important lightning parameters also need to be
There should be a comprehensive and carefully coordinated effort to understand the basic physics of intracloud and cloud-to-ground lightning discharges and their effects on our geophysical environment . This new knowledge should be applied to the development of improved lightning-protection methods.
Several new techniques are now capable of providing much insight into the complex and varied physical processes that occur during a lightning flash. For example, radio interferometry and time-of-arrival methods can be used to trace the three-dimensional development of lightning channels with microsecond resolution. Rockets can be used to trigger, lightning under a thunderstorm, so that many of the physical properties of the discharge and its interactions with structures can be studied in a partially controlled environment.
- The question of how thunderclouds generate electricity has been a fascinating scientific problem for over two centuries, but only in the past decade have cooperative experiments using new experimental techniques provided valuable insights into the complex and varied electrical processes that occur within clouds. Unfortunately, there are no sensors that can determine the charge-size relationship on the smaller cloud particles inside a thunderstorm; thus the data are not adequate to determine which of the many possible mechanisms dominate the generation and separation of charge. In addition to not knowing the charge-size relationship for various cloud particles, it is not known how this relationship evolves with time when there is lightning. The electrical forces on individual elements of precipitation can be several times larger than gravity, but further research is needed to determine whether (and how) these and other electrical effects play a significant role in the formation of precipitation. In view of the successes of recent research, significant new understanding of cloud electricity and lightning can be made by continuing to develop new instruments and by making coordinated in situ and remote measurements of selected thunderclouds. These studies should be complemented by measurements of cloud microphysics and dynamics, by comprehensive laboratory studies, and by theory and numerical modeling.
The complexity of the processes that produce both precipitation and lightning makes
OVERVIEW AND RECOMMENDATIONS 18
it impossible to construct or validate theories of cloud electrification from simple field experiments. It is only through the complementary efforts of comprehensive field observations, laboratory experimentation, and numerical modeling that we can hope to understand the physical processes that are important in thunderstorms. An improved understanding of the major processes that create strong electric fields and their interactions with cloud particles and precipitation might lead to better forecasting of electrical hazards to aviation, forestry, and other outdoor activities.
The first goal of the in-cloud measurements should be to determine the charge-size relationship for various cloud and precipitation particles and the role of screening layers in the upper and lower regions of the storm. The electric current densities that flow above and below the cloud should also be monitored as a function of time. Since the natural storm environment is complicated, laboratory experiments should focus on the detailed physics of mechanisms that appear to be important on the basis of both the incloud measurements and the numerical models. Laboratory experiments should also determine the effects of electric fields on drop coalescence efficiencies and the ability of electrified drops to scavenge charged constituents of atmospheric aerosols. Analyses of the in-cloud and laboratory data could be accelerated through the establishment of a common data base, particularly for theory and numerical modeling efforts.
- Even in fair weather the solid earth and atmosphere are electrified. Thunderstorms have been identified as the dominant generator in the global electric circuit, but many details remain concerning storms as electrical generators and their electrical interactions with their neighboring environment. Lightning and the steady currents above and below thunderclouds play an important role in maintaining an electrical potential between the upper atmosphere and the surface, but the amount and type of lightning and the values of cloud currents that flow to the surface and the upper atmosphere are not well known. The lightning phenomenology and cloud currents may depend on many factors, such as the geographical location of the storm, the season, and the meteorological environment; these dependencies have yet to be determined. The charge transports to the surface under a storm are due to linear and nonlinear field-dependent currents, precipitation and other forms of convection currents, and lightning. Unfortunately, the values of each of these current components and their dependence on the stage of the storm, the lightning-flash frequency, or the local terrain are poorly known. The charge transports to land and ocean surfaces that occur in fair weather, and also to mountainous terrain, need to be determined. With recent progress in the development of satellite lightning sensors and the technology for measuring the electrical effects of storms with rocket-, balloon-, aircraft-, and ground-based sensors, a new attack on this fascinating problem of atmospheric electricity is
There should be an effort made to quantify further the electrical variables that are acting in the global electric circuit and to determine their relationship to the various current components that flow within and near thunderstorms. There is also an important need for theoretical and numerical studies to quantify further the role of thunderstorms as generators in the global circuit.
The establishment of the ionospheric potential, or some other globally representative parameter, as a geoelectrical index that gives an indication of the state of the global circuit would be extremely useful. This index would be the electrical equivalent of the geomagnetic index that has been used for many years to characterize geomagnetic phenomena.
The effects of stratiform clouds and large-scale cyclones on the global circuit also need to be quantified. Once a globally representative parameter that describes the state of the global circuit has been obtained, it can then be related to other remotely observed
OVERVIEW AND RECOMMENDATIONS 19
quantities such as the global lightning flashing rate or directly observed quantities such as the air-earth current or surface electric field.
- Electromagnetic and optical sensors, both on the ground and on satellites, can be used to (1) detect and map
lightning on a regional, national, and global scale and (2) determine, for the first time, how much lightning actually occurs and its geographic distribution as a function of time. With ground-based sensors, it should be possible to determine whether and how the characteristics of individual lightning flashes depend on their geographical location and the storm structure. If a global detection capability were implemented, it would be possible to map and monitor the intensity of lightning storms and to examine the effects of lightning on the global circuit, the ionosphere, and the magnetosphere. When combined with simultaneous spectroscopic measurements, the satellite data could also be used to determine when and where lightning produces significant concentrations of trace gases in the atmosphere. A lightning sensor, capable of measuring lightning flashes during both day and night, should be flown on a geosynchronous satellite at the earliest possible date. The resulting data when combined with those from other sensors and data from ground-based detection networks will provide information that could be used to relate lightning to storm size, intensity, location, rainfall, and other important meteorological parameters.
- Electrical processes in the lower atmosphere and, in particular, within the planetary boundary layer, are important because these, together with global variations, determine the electrical environment of man and the biosphere. Galactic cosmic rays and various radioactive decays produce atmospheric ions that undergo a complex and still only partially understood series of ion-chemical reactions. The composition of the ions is poorly known between the surface and about 50 km, and profile measurements are needed. How the ion characteristics relate to atmospheric aerosols and various trace gases needs to be determined before the bulk electrical properties of the atmosphere can be understood. A significant fraction of the ions attach to atmospheric particles; therefore, smoke and other forms of particulates can significantly affect the electrical properties of the lower atmosphere. Turbulence and convection in the planetary boundary layer play an important role in establishing the vertical distributions of ions, trace gases, and particles. These processes also transport space charge and drive convection currents that alter the electrical properties of the planetary boundary
The clarification of the chemistry of atmospheric ions, their mobilities, and the physics of electrical processes in the troposphere and stratosphere will require further measurements, particularly in determining how these processes are affected by man's activities and natural events . There is also a need for further laboratory measurements and modeling to determine the important chemical reactions and ion composition in the atmosphere.
A number of meteorological research stations in a variety of geographic locations should begin to measure electrical parameters routinely to determine the relationships between electrical and meteorological processes. Vertical profile measurements of electrical properties should be continued in an attempt to determine their relationships to aerosols and trace-gas chemistry. Provisions should be made for the expansion of such synoptic measurements during planned international programs (e.g., the Global Change Programme of the International Council of Scientific Unions, which is currently in the planning stages).
- Recent research has indicated that the mesosphere may not be electrically passive but may, in fact, contain
active electrical generators that are not understood. In addi
OVERVIEW AND RECOMMENDATIONS 20
tion, ground-based and balloonborne measurements have indicated that there is a global electrical response to cosmic-ray and solar variations that is also not understood. The bulk electrical properties of the middle atmosphere are poorly defined, and there is a need to determine the ion composition and chemistry both for quiet conditions and during solar-terrestrial events. There is also new evidence that the electric fields produced by thunderstorms and lightning can produce significant disturbances in the electrical structure of the upper atmosphere and magnetosphere.
The horizontal electric fields that are generated by the ionospheric-wind dynamo and the solar-wind/ magnetospheric dynamo propagate downward to the Earth's surface where they can locally perturb the fair-weather electric field by about 1-2 percent and 20-50 percent, respectively. Horizontal currents in the middle atmosphere and the characteristics of the equalization layer need to be determined in order to understand better the electrical interactions that occur between the upper and lower atmosphere.
To determine (1) the electrical properties of the middle atmosphere, (2) the effects of thunderstorms on ionospheric and magnetospheric processes, and (3) the effects of time variations in the cosmic-ray and energetic solar- particle fluxes on the properties of the global circuit, additional measurements are required. Theoretical investigations and modeling are also important components of such investigations.
Lightning has long been known to be a source of whistlers in the Earth's magnetosphere, and recent spacecraft observations suggest that lightning also generates whistler-mode signals on Jupiter. The questions of just how lightning fields couple to a whistler duct and whether these fields have effects on the ionosphere or magnetosphere are important and need further investigation.
References
Dolezalek, H., and R. Reiter, eds. (1977). Electrical Processes in Atmospheres , Steinkopff, Darmstadt, Germany.
Kessler, E., ed. (1982). Thunderstorms: A Social, Scientific, and Technological Documentary , Univ. of Oklahoma Press, Norman, Okla.
Orville, R. E. , ed. ( 1985 ). Proceedings of the VIIth International Atmospheric Electricity Conference, special issue of J. Geophys. Res. 90
(June 30, 1985).
Volland, H., ed. (1982). Handbook of Atmospherics, Vol. I and II, CRC Press, Inc., Boca Raton, Fla.
http://www.nap.edu/open book/0309036801/htm I/R1. htm I, copyright 1986, 2000 The National Academy of Sciences, all rights reserved
STUDIES IN OEOPHYSICS
The Earth’s Electrical
Environment
Ceophysics Study Committee Ceophysics Research Forum Commission on Physical Sciences,
Mathematics, and Resources National Research Council
NATIONAL ACADEMY PRESS
Washington, D.C. 1986